Considerations when treating lung cancer with passive scatter or active scanning proton therapy
Proton range
In proton therapy, there is a finite range for protons in the patient. The protons stop shortly after the depth of maximum dose deposition—this is referred to as the Bragg peak and is illustrated in Figure 1. Due to this finite range in tissue, the delivered dose from proton radiation therapy is more sensitive to density changes, when compared to external beam photon radiation therapy. It is the finite range of the protons in tissue that enables sparing of nearby healthy structures that is not physically possible in external beam photon radiation therapy. It is also this finite range, and the sensitivity of the quality of the treatment plan to perturbations along the beam path, that make treating lung cancers with protons challenging.
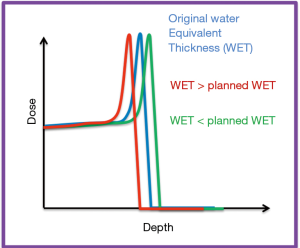
Treatment planning algorithm considerations
One essential component of a successful proton therapy program is the treatment planning system used. Proton treatments in heterogeneous regions have long been known to have larger uncertainties than in homogeneous regions (e.g., lung, supratentorial brain, liver). One consideration is the uncertainty in the range (1). Recommendations have been made to have larger range margins when treating lung (2), which would partly negate the dosimetric benefit of protons. More recently, Monte Carlo simulations of treatment plans have shown that when treating volumes in the lung, the standard pencil beam algorithm in most treatment planning systems is inadequate. The pencil beam algorithm overestimates the dose to the target, and the patient may in fact receive a lower dose than prescribed (3). Similarly, it also overestimates the range of the protons, and the chosen beams may not cover the entire target. The results of these simulations have been confirmed by end-to-end tests; for sites participating in National Cancer Institute (NCI) supported clinical trials on the use of proton therapy for lung cancer, a heterogenous phantom representing a typical thoracic patient is irradiated and the accuracy of the sites ability to treat a volume in a heterogenous volume is determined. Accurate irradiation of this phantom is required if sites wish to enroll patients on clinical trials. An analysis of these phantom irradiations has shown that fewer than 60% of the sites that irradiate the phantom pass the requirements, with pencil beam algorithms over-estimating the dose to the target by up to 46%. When the treatment planning system was changed to a Monte Carlo based system from the standard pencil beam algorithm, disagreements between the measured and predicted doses were resolved. This has resulted in the strong recommendation that when treating in the lung, pencil beam algorithms should never be employed (4). For any type of proton treatment in the lung, dose accuracy is greatly improved if advanced dose calculation algorithms, such as Monte Carlo methods, are used.
Patient motion
Patient motion is assessed during pretreatment imaging. This can be done through a series of one or more four-dimensional computed tomography (4D-CT) imaging studies. In a 4D-CT the breathing pattern of the patient is monitored and several CT scans are acquired per bed position. When the images are reconstructed, each image is associated with a specific phase or amplitude of the breathing cycle and the tumor trajectory can be visualized through all breathing phases. The internal motion of the tumor can be captured and the magnitude of the motion can be quantified. The 4D-CT is used to characterize the tumor motion due to the patient breathing and this informs the creation of the internal target volume (ITV) (5), from which the treatment plan is generated. From the 4D-CT, other secondary scans can be derived and used in the treatment planning process. In proton therapy treatment planning is typical for the average intensity projection (AIP) image to be used. A conservative approach is for the ITV to be overwritten with Hounsfield units (HUs) slightly denser than water (6) on the AIP. The success of this approach has more recently been evaluated in the active scanning environment, and it was found that applying an over-write of the water equivalent path length (from the beams eye view) in the ITV performed better than a uniform over-ride of the ITV (7).
The interplay effects
The temporal dependences of the treatment delivery, combined with the breathing motion of the patient have the potential to result in the planned dose and the delivered dose not being in agreement due to the so-called interplay effect. The interplay effect has been studied for many years for complex intensity modulated radiation therapy (IMRT) treatment plans (8,9), for proton double scattering plans (6,10) and, more recently for active scanning treatment plans (11-14). Early geometric studies suggested that dose variations of 10% would be seen due to the interplay effect with scanned proton fields and target motion (15). Four-dimensional Monte Carlo treatment planning studies have been performed to demonstrate that for active scanning systems, ignoring target motion can result in a decrease of dose homogeneity to the target, resulting in a higher maximum dose and a lower minimum dose. In one example, where a patient had a breathing amplitude of 30 mm peak-to-peak, the maximum dose to the tumor increased to 140% of the prescribed dose and the minimum dose to the tumor decreased to 44% of the prescribed dose (13). These results were for a single fraction however, and do not include the effects of averaging over multiple fractions in a conventional treatment regimen. Most studies on the interplay effect ignore baseline shifts. Baseline shifts in breathing, where the patient is breathing deeper or shallower than on the day of simulation, have been reported (16) and shown in simulations to decrease the dose to the clinical target volume (CTV). The dose to the CTV can also be impacted by variations in the patients breathing cycle duration. The dose homogeneity in the presence of baseline shifts and variations in breathing cycle improved with layer repainting and an increase in the number of fractions (17).
Proton therapy delivery systems: similarities and differences
How the proton field is shaped and directed at the patient can impact the temporal dependence of the delivery. The main equipment dependencies can be considered in one of two main categories for delivery: passively scattered proton therapy and active scanning. A third category, uniform scanning (US), may be thought of as a hybrid of active scanning and passively scattered proton therapy. Historically, all proton therapy centers used double scattering; now, many centers employ active scanning exclusively and the vast majority of new facilities under construction will use active scanning as well. An illustration of the three systems is shown in Figure 2.
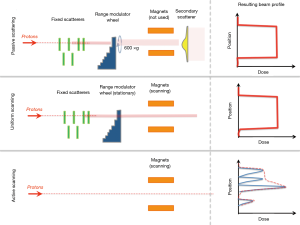
Passive scattered proton therapy (PSPT)
In passive scattering, the proton beam is spread out to be a uniform beam (laterally) through single or double scattering. Single scattering may be used for smaller field sizes—to have larger uniform field sizes a double scattering system is required. Most double scattering systems can produce a uniform field distribution for maximum field sizes of up to 25 cm diameter. The edges of the beam can then be shaped laterally by collimators to shape the radiation field that impinges on the patient. A spinning range modulator wheel allows for the beam energy to vary with time and the result is that there is a spread in the energies of the protons, resulting in a dose distribution that is a spread out Bragg peak. The different energies are not all delivered simultaneously, but all of the energies are delivered in a single cycle of the range modulator wheel, which rotates at a typical frequency of 10 Hz. As such, the temporal variance of the delivery of the energies is much less than typical breathing cycles. In treatment planning for PSPT, the specific beam angles will be selected (e.g., gantry angle, couch angle) along with an appropriate range and modulation of the spread out Bragg peak to ensure target coverage. The apertures are automatically created with a uniform margin to the target and manually edited if desired. The range compensators are also automatically generated. Once the plan has been completed, the hardware can be fabricated. The hardware fabrication for PSPT necessitates the use of an in-house machinist, materials (brass, Lucite, wax) and the time to fabricate the devices.
Active scanning
In active scanning (often called pencil beam scanning or spot scanning) a focused beam of protons of variable intensity and energy is magnetically scanned across the field. The diameter of the individual spots varies as a result of energy, ranging from ~4 mm spot sigma to 10 mm sigma. The shape of the spots is also a function of the beam steering in the system. A good description of active scanning systems can be found in Kooy et al. (18).
This scanning technique allows for conformal dose in the lateral directions (x, y) without using additional hardware. Brass apertures are not required for active scanning, as the lateral extent of the treatment field can be maintained by choosing appropriate spot positions. In addition, the ability to control the energy (and therefor the depth of penetration of the protons) results in not needing field specific range compensators and the resulting beams can conform to the proximal and distal shapes of the target. The maximum field size of these systems is up to 30 cm × 40 cm. Unlike treatment planning for PSPT, the treatment planning for active scanning is too complex to do without advanced treatment planning systems. For every beam, there are spots of varying weights that can be positioned anywhere within the patient. The complexity of the treatment plan is dealt with by using inverse treatment planning systems where spots are positioned to achieve the required dose to the target and spare nearby organs at risk. In this manner, the treatment planning process closely resembles the treatment planning process for IMRT. Unlike IMRT, there is the additional option to uniformly cover the treatment volume with every beam (single field uniform dose) or to have complimentary fields, where the entirety of the target is covered by the multiple fields in the treatment plan, but not by a unique field in the treatment plan (e.g., multi-field optimization).
US
US is a third modality that is available. In many ways, US may be described as a hybrid of passive scattering and active scanning. In US, protons of a single energy are delivered at one time, as is done in active scanning and the entire energy range is delivered through many (tens) energy layers delivered in one setting. Once one energy layer is delivered, the energy layer is switched and the next energy layer is delivered. Each energy layer reaches a unique maximum depth in the patient and the spacing between the energy layers is determined by the width of the Bragg peak of the previous layer. Energy switching takes 0.5–1.0 second. To achieve a flat field a broad pencil beam (typical spot sigma of 5 cm) is magnetically steered across the entire field of treatment, resulting in a large uniform field. Unlike in the case of active scanning, the beam is not turned off between positions—it is continuously scanned across the treatment field at rates of 30 Hz in the fast direction and 3 Hz in the slow direction. As in double scattering, brass apertures to shape the field laterally are required as are range compensators to shape to shape the distal portion of the field. While the proton beam is magnetically scanned in US, the scanning is a constant raster scanning across the entirety of the uniform field size and all lateral beam shaping is done using the brass apertures.
An illustration of the three discussed dose delivery options is shown below in Figure 2. Highlighted are the differences in treatment hardware and the direction of the scanning beams.
The equipment differences discussed above do not change the dose a stationary tumor receives. In the case of active scanning, a dosimetric advantage exists because the dose can be more conformal on the proximal and distal ends of the target, better sparing healthy tissues and organs at risk. An additional advantage is the ability to easily create treatment plans of multiple dose levels to different parts of the target volumes, thereby achieving more refined clinical goals. The equipment differences above, do however, result in different temporal variations in how the dose is delivered and may result in dose differences in disease sites with organ motion (e.g., lung, liver).
Time dependence of passive scattering, active scanning and US delivery of proton therapy
This time dependence is not necessary to consider when patient motion is negligible, but should be considered in treating lung cancers. The three modalities can be ordered from least variable with time to most variable with time. The least time dependent of the three modalities is PSPT. For PSPT, all proton energies are delivered with every rotation of the modulation wheel (0.1 second per rotation). The most time dependent of the three modalities is active scanning where each energy layer is scanned through and then layer-switching pauses (on the order of 1–5 seconds) occur between each energy layer. US falls between the two categories. There is very little time dependence in the lateral direction of the beam (as in PSPT); however, as in active scanning, only one energy layer is delivered at a time and there is an additional pause for energy layer-switching.
Motion management
There are several strategies for dealing with patient motion. A common approach is to first understand the magnitude of the motion. This can be done through use of a 4D-CT. In many instances, it has been found that motion amplitude greater than 1 cm peak-to-peak is a contraindication for treatment with proton therapy.
Once the decision to treat the patient using proton therapy has been made, there are methods to minimize the effects of motion on the dose to the tumor. Two distinct options include (I) gating the delivery of the beam and, in the case of active scanning, (II) layer repainting.
Gated delivery
In gated delivery, the beam is only on, and radiation is only delivered when the patient is in a predetermined portion of the breathing cycle. The delivered radiation is in synchrony with the patient’s breathing on the day of treatment. This requires monitoring the patient during the treatment and the ability to automatically turn the proton beam off and on. For passive scatter proton therapy, this has been implemented (19). For active scanning, gating has been proposed but is not commercially available. Note that gating treatment can also be performed with the patients holding their breath for a short period of time, e.g., 15–25 seconds, if their medical condition allows.
Repainting strategies
Layer repainting was first suggested as a method to mitigate the interplay effect for active scanning (20). In layer repainting, the dose from a single energy (layer) is broken into multiple equally weighted segments and the energy layer is delivered multiple times. Another repainting strategy proposed is volumetric repainting (21), where the entire field is treated to a dose of 1/N times the desired dose and this is repeated N times.
The challenge of managing motion is not unique to proton therapy. For external beam photon therapy, many considerations must also be taken into account when choosing appropriate treatment planning and motion management strategies (22).
Conclusions
Treating lung cancer using proton therapy has the potential for great dosimetric benefits due to the proximity of nearby healthy organs. Due to the physical characteristics of protons, doses to tumors may be escalated while normal tissue is spared. As a result, proton therapy has the potential to improve patient outcomes.
For many disease sites, how the proton fields are shaped and delivered does not impact the quality of the treatment. For lung cancer, and any site where organ motion is a consideration, one must be aware of potential interplay between the motion of the target and the delivery of the radiation field.
In proton therapy, as we move towards more complex treatment delivery systems, state of the art treatment planning systems (e.g., Monte Carlo based) will ensure that the designed treatment plan for the patient matches the radiation dose the patient receives.
Acknowledgements
None.
Footnote
Conflicts of Interest: The authors have no conflicts of interest to declare.
References
- Paganetti H. Range uncertainties in proton therapy and the role of Monte Carlo simulations. Phys Med Biol 2012;57:R99. [Crossref] [PubMed]
- Schuemann J, Dowdell S, Grassberger C, et al. Site-specific range uncertainties caused by dose calculation algorithms for proton therapy. Phys Med Biol 2014;59:4007. [Crossref] [PubMed]
- Grassberger C, Daartz J, Dowdell S, et al. Quantification of proton dose calculation accuracy in the lung. Int J Radiat Oncol Biol Phys 2014;89:424-30. [Crossref] [PubMed]
- Taylor PA, Kry S, Followill D. Pencil beam algorithms are unsuitable for proton dose calculations in lung. Int J Radiat Oncol Biol Phys 2017;99:750-6. [Crossref] [PubMed]
- Wambersie A, Landgerg T. ICRU report 62: prescribing, recording and reporting photon beam therapy. Bethesda, MD: ICRU, 1999.
- Kang Y, Zhang X, Chang JY, et al. 4D Proton treatment planning strategy for mobile lung tumors. Int J Radiat Oncol Biol Phys 2007;67:906-14. [Crossref] [PubMed]
- Botas P, Grassberger C, Sharp G, et al. Density overwrites of internal tumor volumes in intensity modulated proton therapy plans for mobile lung tumors. Phys Med Biol 2018;63:035023. [Crossref] [PubMed]
- Yu CX, Jaffray DA, Wong JW. The effects of intra-fraction organ motion on the delivery of dynamic intensity modulation. Phys Med Biol 1998;43:91-104. [Crossref]
- Seco J, Sharp G, Turcotte J, et al. Effects of organ motion on IMRT treatments with segments of few monitor units. Med Phys 2007;34:923-34. [Crossref] [PubMed]
- Engelsman M, Rietzel E, Kooy HM. Four-dimensional proton treatment planning for lung tumors. Int J Radiat Oncol Biol Phys 2006;64:1589-95. [Crossref] [PubMed]
- Seco J, Robertson D, Trofimov A, et al. Breathing interplay effects during proton beam scanning: simulation and statistical analysis. Phys Med Biol 2009;54:N283-94.
- Bert C, Grözinger SO, Rietzel E. Quantification of interplay effects of scanned particle beams and moving targets. Phys Med Biol 2008;53:2253. [Crossref] [PubMed]
- Grassberger C, Dowdell S, Lomax A, et al. Motion interplay as a function of patient parameters and spot size in spot scanning proton therapy for lung cancer. Int J Radiat Oncol Biol Phys 2013;86:380-6. [Crossref] [PubMed]
- Grassberger C, Dowdell S, Sharp G, et al. Motion mitigation for lung cancer patients treated with active scanning proton therapy. Med Phys 2015;42:2462-9. [Crossref] [PubMed]
- Bortfeld T, Jokivarsi K, Goitein M, et al. Effects of intra-fraction motion on IMRT dose delivery: statistical analysis and simulation. Phys Med Biol 2002;47:2203. [Crossref] [PubMed]
- Shirato H, Suzuki K, Sharp GC, et al. Speed and amplitude of lung tumor motion precisely detected in four-dimensional setup and in real-time tumor-tracking radiotherapy. Int J Radiat Oncol Biol Phys 2006;64:1229-36. [Crossref] [PubMed]
- Kraus KM, Heath E, Oelfke U. Dosimetric consequences of tumour motion due to respiration for a scanned proton beam. Phys Med Biol 2011;56:6563. [Crossref] [PubMed]
- Kooy HM, Clasie BM, Lu HM, et al. A case study in proton pencil-beam scanning delivery. Int J Radiat Oncol Biol Phys 2010;76:624-30. [Crossref] [PubMed]
- Lu HM, Brett R, Sharp G, et al. A respiratory-gated treatment system for proton therapy. Med Phys 2007;34:3273-8. [Crossref] [PubMed]
- Phillips MH, Pedroni E, Blattmann H, et al. Effects of respiratory motion on dose uniformity with a charged particle scanning method. Phys Med Biol 1992;37:223. [Crossref] [PubMed]
- Bernatowicz K, Lomax A, Knopf A. Comparative study of layered and volumetric rescanning for different scanning speeds of proton beam in liver patients. Phys Med Biol 2013;58:7905. [Crossref] [PubMed]
- Brandner ED, Chetty IJ, Giaddui TG, et al. Motion management strategies and technical issues associated with stereotactic body radiotherapy of thoracic and upper abdominal tumors: A review from NRG oncology. Med Phys 2017;44:2595-612. [Crossref] [PubMed]