Stereotactic body radiation therapy for empirically treated hypermetabolic lung lesions: a single-institutional experience identifying the Charlson score as a key prognostic factor
Introduction
Stereotactic body radiation therapy (SBRT), also known as stereotactic ablative radiotherapy (SABR), is a method for delivery of high doses of radiation in relatively few fractions (1,2). Prospective data has demonstrated encouraging results in medically inoperable non-small cell lung cancer (NSCLC), resulting in further questions regarding its role in medically operable patients and those with central tumors (3,4). Most patients have a cancer diagnosis confirmed via biopsy, and the resulting histological data has further refined the role for SBRT (5).
Guidelines for selection of patients with lung cancer for surgery rely on fitness for surgery (age, pulmonary function, cardiovascular fitness, nutritional status, and performance status) and operability (6). Though some patients with poor baseline pulmonary function may still benefit from surgery, Sancheti et al. noted 3-year survival of only 59% in such “high-risk” patients, compared to 76% in standard risk patients (7,8). Obtaining tissue biopsy of a potential tumor is generally undertaken via computed tomography (CT)-guided transthoracic lung biopsy, which carries approximately a 5.7% risk of a major complication (9). Other studies have noted an overall complication rate of 39.1% (10-13), resulting in relative contraindications to biopsy such as forced expiratory volume in 1 second (FEV1) <35% predicted (14,15).
Chronic obstructive pulmonary disease (COPD) is a common disorder with the same principal risk factor as lung cancer: cigarette smoking (16,17). For this reason, COPD is prevalent among lung cancer patients and serves as a major factor limiting pulmonary function and serving as a contraindication to biopsy. While COPD’s potential role in NSCLC outcomes has been explored, a more holistic assessment of a patient’s health status is needed (18,19). The Charlson score and the associated Charlson comorbidity index (CCI) have been well-studied and validated (20-22). They have been used in a range of oncologic contexts and have demonstrated prognostic value, including in lung cancer patients (23-28). To our knowledge, the Charlson score has never been utilized to predict survival in unbiopsied, empirically treated cancer patients.
Empirically treating cancer requires some degree of certainty that the suspicious lesion is malignant. Physicians can surveil the lesion with imaging to assess for growth, but positron emission tomography (PET) offers the unique opportunity to assess for hypermetabolic activity. PET is increasingly being used in oncology, resulting in potential upstaging of a tumor and a change in treatment in approximately 10–15% of cases (29,30). Though false positives can occur, PET has been found to have higher sensitivity for diagnosis than some conventional imaging (31-34). Due to the high prevalence of solitary pulmonary nodules of undetermined significance, there is an abundance of clinical data regarding the utility of PET in distinguishing between benign and malignant lesions (35). Therefore, PET imaging is a valuable tool that can assist in patient selection for potential empiric SBRT, regardless of the standardized uptake value (SUV) cutoff used to diagnose hypermetabolic lesions.
There are few studies in the literature concerning unbiopsied lung SBRT, but results have been encouraging (36-41). Though none of the studies included more than 115 patients, none of the analyses reported more than three cases of grade ≥3 toxicity. Further, multiple studies have reported local control rates of greater than 90%. These analyses have included a variable number of patients and have utilized a diverse set of inclusion criteria, pointing towards a role for further single-institutional study. Such a study could also report on whether the generally low rates of toxicity with SBRT for early stage NSCLC might be increased in empirically treated patients who generally suffer from worse overall health status (42-44).
This study seeks to present a single institutional experience with empirically treating non-metastatic NSCLC demonstrating hypermetabolic activity on PET imaging. The authors aim to present data regarding overall survival (OS), local control, and toxicity and determine factors predictive for OS and local control. By considering a range of factors, including biologically effective dose (BED), pre-treatment SUV, the Charlson score, and lesion size, we hope to delineate a set of prognostic factors to assist patients and providers in shared decision-making regarding the utility of empiric SBRT for NSCLC. In particular, the authors aim to demonstrate that the Charlson score is a useful prognostic indicator in empirically treated lung cancer patients. We present the following article in accordance with the STROBE reporting checklist (available at http://dx.doi.org/10.21037/tlcr-20-469).
Methods
Patient cohort
The patient cohort consisted of presumed non-metastatic NSCLC that was empirically treated with SBRT. Specific inclusion criteria required patients to have a hypermetabolic pulmonary lesion noted on PET imaging but no pathological evidence of NSCLC. Generally, SUV ≥2 was considered the threshold for a lesion being hypermetabolic; however, in rare cases, treatment might be undertaken if a lesion demonstrated other concerning features (e.g., continued growth on CT with an SUV of 1.8). Patients with another known metastatic tumor that could have resulted in the lung lesion were excluded. Patient data was retrospectively reviewed, and patients without sufficient information to determine Charlson and Karnofsky performance status (KPS) scores were excluded, as these scores were deemed critical to assessing patients’ overall health statuses. Patients with stage 3 disease were not excluded from the study. Though SBRT is often not the standard treatment for stage 3 NSCLC, at this institution, some stage 3 patients refused chemotherapy or other treatment options and were treated with SBRT. These patients were generally T stage 1 or 2 disease with significant medical comorbidities and nodal spread of disease. Since they comprise a non-trivial fraction of empiric SBRT, they were included in the study. Patients with T stage ≥3, however, were excluded because these tumors are generally too large for the use of SBRT. Patients from March 2008–June 2016 were considered for inclusion.
Ninety-one such treatments in 90 unique patients met all inclusion criteria from an initial set of approximately 420 cases of SBRT for NSCLC. To assess the patient’s overall health status, the following data were obtained: KPS, the reason for the lack of biopsy, and the Global Initiative for COPD (GOLD) classification. Patient comorbidities were assessed to compute a Charlson score and subsequently the CCI 2-year survival estimate. The Charlson score was computed by identifying key comorbid conditions and summing their respectively assigned weightings (in parentheses): acute myocardial infarction [1], congestive heart failure [1], peripheral vascular disease [1], cerebrovascular disease [1], dementia [1], chronic lung disease [1], rheumatic disease [1], peptic ulcer [1], mild liver disease [1], mild to moderate diabetes [1], diabetes with chronic complications [2], hemiplegia or paraplegia [2], kidney disease [2], malignant tumor [2], moderate to serious liver disease [3], solid and metastatic tumor [6], and AIDS [6]. Chart review was principally conducted by a single investigator to minimize bias.
The lesion was further described according to its location (central vs. peripheral), longest dimension, and pre-treatment SUV. A central location was defined as within 2 cm of the carina. Prior treatment history [chemotherapy, thoracic surgery, and thoracic radiotherapy (RT)] was noted, and the cancer staging prior to treatment was recorded. This study was exempt by the institutional review board and the informed consent due to its retrospective nature. And all procedures performed in this study were in accordance with the Declaration of Helsinki (as revised in 2013).
Treatment planning, localization, and delivery
Where applicable, previous radiation therapy treatment planning files, including DICOM-RT dose files, were obtained and imported into the treatment planning system for evaluation of prior dose to organs-at-risk. Patients were immobilized using a full-body vacuum bag system for position stabilization and consistency. Serial CT scans were taken (free-breathing, inhale, and exhale) for treatment planning purposes and to assess the motion of the target during the breath cycle and generate an internal-target-volume (ITV) margin around the gross tumor volume (GTV). Dose was prescribed to the planning target volume (PTV) which was defined as the ITV plus several mm of margin to account for uncertainties in imaging and localization. In general, a 3D conformal treatment planning approach with non-coplanar gantry angles was used to minimize dosimetric overlap of entrance and exit portals. Intensity-modulated RT and volumetric-modulated arc therapy were considered but not used in this context. SBRT was delivered using a 6MV photon beam on a linear accelerator with a 2.5–4 mm width multi-leaf collimator for custom shaping of portals.
On-board cone-beam CT (CBCT) with 4D capabilities was used prior to treatment, and a 6D robotic couch assisted in the alignment of the patient and localization of the target to the planning CT. CBCT would be repeated several times during treatment to correct any intra-fraction motion of the patient or target.
Dose delivered to the tumor was generally 40–60 Gy, with only two treatments involving lower doses. All treatments involved 3–5 fractions of therapy. BED was calculated using the formula dose * (1 + dose per fraction/alpha beta ratio), where the alpha beta ratio of 10 was used in this case.
Endpoints
Patients received follow-up imaging generally 2–3 months after completion of treatment. Seventy-seven treatments had follow-up with PET (85%), with additional patients mostly followed by serial CT scans. Post-treatment SUV was recorded when available, despite it not being a standard follow-up measure at all institutions. Key endpoints included: OS, progression-free survival, local control, and radiographic response. Tumor recurrence was delineated as local, regional, or distant failure, and the time of failure was recorded as the date of the imaging study on which the recurrence was noted. Radiographic response was graded as complete, partial, stable, or progressive according to the RECIST criteria. Toxicity was recorded according to the Common Terminology Criteria for Adverse Events v5.0. Follow-up was conducted primarily via radiation oncology and pulmonary physicians.
Statistics
Statistical analysis was conducted primarily via univariate analysis, Cox proportional hazards analysis, and Kaplan-Meier analyses. A P value of <0.05 was deemed to be statistically significant; otherwise, any result recorded was only a potential statistical trend. Univariate analyses were conducted via two-tailed t-tests. Cox proportional hazards analysis was conducted to consider prognostic factors for the endpoints of OS, progression-free survival, and local control. Factors cut from analysis included dose and the status of prior treatments (chemotherapy, thoracic surgery, and thoracic RT). Dose was eliminated because of collinearity with BED, and fewer than five patients had evidence of chemotherapy, thoracic surgery, or thoracic RT. Such a small subset of patients would be unlikely to demonstrate statistical significance. Kaplan-Meier analysis was conducted to stratify the patient set into subgroups to consider factors predictive of OS. The Kaplan-Meier method was also used to estimate 24- and 36-month rates of local control, OS, and progression-free survival.
Results
Patient cohort
Ninety-one treatments for ninety unique patients met inclusion in the study. Forty-one patients were male (46%) while 49 were female (54%). Patients were generally advanced in age, with a median age of 77.9 years at the start of treatment. Median KPS was 80. The reason for the lack of a biopsy was most commonly due to COPD (46%), patient refusal (24%), and concern about multiple medical comorbidities (11%). In the cases of patient refusal, a biopsy was deemed to be feasible and medically indicated, but the patient elected not to undergo the procedure. Less common reasons included: morbid obesity, advanced age, and the location of the tumor. There were six cases of an attempted but non-diagnostic biopsy. Treatment targets were fairly evenly distributed between the right upper lobe (27.5%), right middle lobe (17.6%), right lower lobe (11.0%), left upper lobe (26.4%), lingula (3.3%), left lower lobe (10.0%), and the pulmonary hilum (4.4%).
The median pack years was 50, consistent with the high frequency of COPD. The median GOLD score was 1, with 53 patients having recorded GOLD scores ≥1, 42 patients with GOLD scores ≥2, and 23 patients with GOLD scores ≥3. Results of pulmonary function testing were recorded when available, and the median FEV1 as a fraction of the predicted value was 63%. The FEV1 divided by the forced vital capacity (FVC) was a median 0.65, and the total lung capacity was a median 100%.
The median Charlson score was 7, with a median 2-year survival projection of only 55%. Twenty-three patients had a Charlson score ≥9, and only 14 patients had a Charlson score <6. Lesions were mostly peripheral (67%) but with a significant minority of central tumors (33%). The median longest dimension was 2 cm, with a median pre-treatment SUV of 4.5. Most tumors were stage 1 (68%) or 2 (24%), with only 8% of tumors at stage 3 at the time of treatment. Thirteen treatments were for lesions with diagnosed nodal spread. Patients were treated with a median 60 Gy in 4 fractions and a median BED of 132 Gy (Table 1).
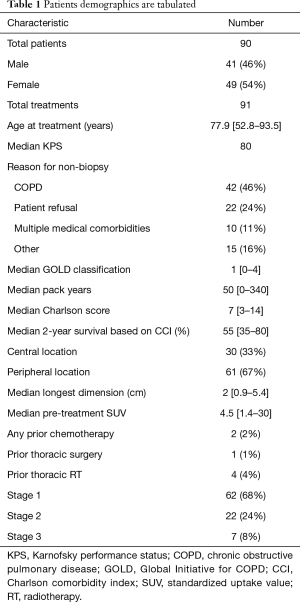
Full table
Endpoints
Patients had a median follow-up time of 12.9 months, and 85% were followed via PET imaging (Table 2). Forty-nine patients received at least two PET scans after completion of therapy. Median post-treatment SUV was 1.5, and median SUV on the second follow-up scan was 1.55. Fifty-four patients (59%) remained alive at the time of chart review, with 41% deceased. Median OS was 14.3 months, and progression-free survival was 9.4 months (Figure 1). Two-year OS via the Kaplan-Meier method was 65.4%, and 2-year progression-free survival was 44.8%. Local control was 92%, with only 7 instances of local failure. Two-year local control was 93.1%, and 3-year local control was 91.3%. This was demonstrated via the Kaplan-Meier method in Figure 2. Median time to local failure was 11.2 months. Regional failure occurred in 16 cases (18%) at a median 5.6 months, and distant failure was noted in 17 instances (19%) at a median 5.8 months. Complete radiographic response was noted after 48% of treatments. Partial response, stable disease, and progressive tumor were found in 37%, 12%, and 2% of cases.
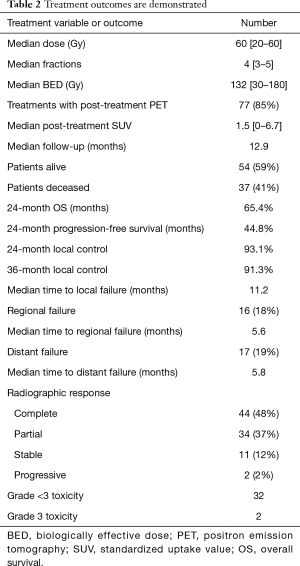
Full table
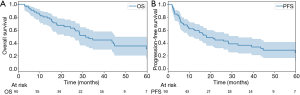
Tumors with longest dimension ≥2 cm demonstrated decreased OS (11.5 months) relative to tumors <2 cm in longest dimension (15.8 months), but this result was not found to be statistically significant on univariate analysis (P=0.11). Cox proportional hazards analysis was conducted to assess for factors predictive of OS, progression-free survival, and local failure (Figure 3). No factors were found to be predictive of progression-free survival or local control. High Charlson score trended towards significance for decreased OS [hazard ratio 1.29 (1.00–1.68)].
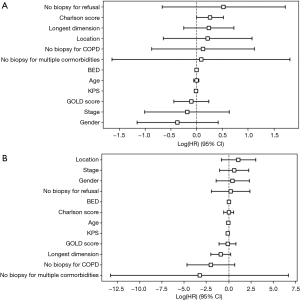
Kaplan-Meier analysis was utilized to stratify the patient cohort into subgroups (Figure 4). Patients with Charlson score ≥9 demonstrated a statistically significant decrease in OS, relative to patients with scores <9 (P=0.04). Thirty-six-month OS was 0% for patients with Charlson score ≥9, and it was 50.3% for patients with Charlson score <9. Additionally, patients treated with BED ≥120 Gy trended towards improved OS relative to patients treated with lower doses (P=0.08). Patients with BED ≥120 Gy had a median OS of 18.0 months, compared to 7.5 months for BED <120 Gy. Similarly, 36-month OS was 50.5% for patients with BED ≥120 Gy, but it was only 31.6% for patients with BED <120 Gy. On univariate analysis, BED ≥120 Gy was a statistically significant predictor of improved OS (P=0.001). On the other hand, BED ≥100 Gy failed to demonstrate improved OS on Kaplan-Meier analysis (P=0.45). Patients with pre-treatment SUV ≥4 demonstrated decreased OS relative to patients with initial SUV <4 (11.0 vs. 15.0 months), but Kaplan-Meier analysis failed to demonstrate statistical significance for this observation (P=0.13).
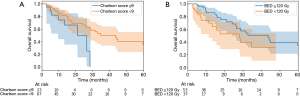
Patients with stage 3 disease were also considered separately, but results were generally similar to the other patients in the study. Four of the seven patients refused the biopsy, and the median Charlson score was 9. Median pre-treatment SUV was 8.0, and treatment delivered a median BED of 112.5 Gy. None of the patients experience local failure, and the median OS was 8.1 (mean: 13.6) months. Finally, only one of these patients reported further disease progression.
Toxicity
Toxicity in this study was minimal. There were 32 instances of grade <3 toxicity among all treatments and only two cases of grade 3 toxicity. No grade >3 toxicity was reported. Both grade 3 toxicities recorded were for fatigue. The most common grade 1 and 2 symptoms were dyspnea, fatigue, and cough, with 11, 10, and 7 cases, respectively. No grade ≥2 radiation pneumonitis was reported.
Discussion
Overall, this study demonstrates encouraging results regarding the use of empiric SBRT for presumed NSCLC in patients without pathological diagnosis. Thirty-six-month local control was high at 91.3%, which is comparable to the involved lobe control rate of 90.6% reported by Timmerman et al. for SBRT in inoperable early stage lung cancer (45). Toxicity was also minimal, with no grade ≥2 pneumonitis and only 2 instances of grade 3 morbidity. Due to the high prevalence of COPD and other major medical comorbidities in the cohort, this result points to the safety of SBRT in the treatment of patients with poor baseline lung function.
Relatively poor OS was noted in this patient cohort, at a median 14.3 months. This result is not unexpected in the context of the high median Charlson score (7), high GOLD scores (42 patients with score ≥2), and a median CCI 2-year survival estimate of only 55%. One of the strengths of this study is this thorough characterization of the patient cohort’s overall health status, and it demonstrates how poor these patients’ survival outcomes likely are at baseline. It also demonstrates how these additional factors should be considered in SBRT patient selection, as the commonly used KPS score was an encouraging median 80 in this cohort. Though useful, KPS does not completely encompass a patient’s health status.
Six other studies of SBRT in unbiopsied lung cancer were identified for comparison. Harkenrider et al. analyzed a set of 34 patients treated at two universities, demonstrating 97.1% local control at a median 16.7 months of follow-up and a 2-year OS of 85%. Predictive factors were not identified, and 3 grade 3 chronic toxicities were reported (36). Hasan et al. considered a larger set of 101 cases, and local control was 94%, with a median 14 months of follow-up. Two grade 3 cases of dyspnea were observed, and pre-treatment SUV >4.1 was identified as a significant predictor of disease progression (37). Two small studies of 25 and 37 patients with stage 1 presumed lung cancer patients reported similar efficacy and tolerability (38,40). Yoshitake et al. considered a larger set of 88 patients and reported results consistent with other studies (41). Inoue et al. studied the largest single set of clinically diagnosed primary lung cancer, with 115 unique patients across 12 institutions. They observed that tumors with size ≤20 mm demonstrated improved 3- and 5-year OS rates (39).
The present study uniquely contributes to the literature, as it is one of the largest single institutional study concerning empirically treated presumed NSCLC. The key outcomes of local control and toxicity were similar to previous studies, but OS was lower, as previously discussed. This analysis is unique in that it included seven cases of stage 3 disease, which did not demonstrate decreased rates of local control. Additionally, this study is the first to consider the Charlson score as a predictor of OS. It was the only factor to trend towards statistical significance on Cox proportional hazards analysis, and the Kaplan-Meier method showed improved OS with scores <9. This would indicate that the dominant factor predicting OS in this relatively ill patient cohort outcomes is overall health status. Additionally, it demonstrates the utility of the Charlson score in this context.
Three tumor-specific factors merit further study for their potential influence on OS in this context: BED, tumor size, and pre-treatment SUV. This is the first study to demonstrate an OS benefit with increased BED (≥120 Gy) on univariate analysis and a corresponding trend on Kaplan-Meier analysis. Interestingly, this is a higher threshold than previous studies noting improved local control with BED ≥100 Gy (46). Treatments with BED ≥100 failed to show improved OS in this study, but this may be due to the low utilization of such low dose treatments since only 6 treatments involved BED <100 Gy. This distinction may be a potential avenue for further study. Tumor size ≤2 cm has previously demonstrated improved OS, and this study noted a non-statistically significant decrease in OS (11.5 vs. 15.8 months) in tumors with longest dimension >2 cm (39). Finally, pre-treatment SUV ≥4 demonstrated a trend towards decreased OS (11.0 vs. 15.0 months), similar to the findings of Hasan et al. (37).
Three large studies were identified that compared outcomes after SBRT between biopsied and unbiopsied lung cancer patients. Takeda et al. compared a set of 58 clinically diagnosed lung cancer patients with 115 pathologically diagnosed lung cancer patients. They found that treatment outcomes were very similar between the two groups, and few benign lesions were included in the clinically diagnosed patient subset (47). A small study from Haidar et al. reported similar results (48). The largest comparison study was conducted by Verstegen et al. comparing 209 pathologically diagnosed tumors to 382 clinically diagnosed patients (49). The study only included stage 1 patients, but no significant differences were found in OS and local control rates.
In light of these findings, it is apparent that SBRT for unbiopsied, presumed NSCLC is well-tolerated and efficacious. Nonetheless, stringent guidelines for patient selection do not exist. Pre-test probabilities ranging from 65% to 85% have been postulated as thresholds for offering empiric SBRT, and distinct thresholds for defining PET-avidity are also in use (50). This study offers a new perspective on this question and provides evidence that patients with BED ≥120 Gy, tumors with longest dimension ≤2 cm, and pre-treatment SUV <4 might experience improved OS.
Limitations of this study include the relatively short follow-up of 12.9 months. This can partly be explained by the poor OS in this patient cohort. Additionally, it is possible that this patient population of individuals without a biopsy consists largely of patients with poor medical follow-up, as 24% of the patients in this study refused the biopsy. Even so, more extensive follow-up might have allowed for further important conclusions to have been drawn. Also, a larger patient cohort might have allowed for more complete characterization of the role of SUV and BED in patient outcomes. Finally, the use of an age-adjusted CCI may provide a different angle for additional study.
Conclusions
Though pathologic evidence for NSCLC is recommended, many patients do not receive a biopsy, posing a question regarding the use of empiric SBRT. Treatment for presumed NSCLC is efficacious for non-metastatic disease, and even the presence of multiple medical comorbidities does not appear to adversely affect toxicity. OS appears to be chiefly influenced by overall health status, and the Charlson score demonstrates utility in predicting OS in these patients. Patients with lesions <2 cm and with pre-treatment SUV <4 may experience improved OS, and providing therapy with BED ≥120 Gy may also improve OS. Even when meeting these thresholds is not possible, patients will still likely benefit from empiric SBRT.
Acknowledgments
Funding: None.
Footnote
Reporting Checklist: The authors have completed the STROBE reporting checklist. Available at http://dx.doi.org/10.21037/tlcr-20-469
Data Sharing Statement: Available at http://dx.doi.org/10.21037/tlcr-20-469
Peer Review File: Available at http://dx.doi.org/10.21037/tlcr-20-469
Conflicts of Interest: All authors have completed the ICMJE uniform disclosure form (available at http://dx.doi.org/10.21037/tlcr-20-469). Dr. ROK reports that the wife of Dr. ROK is a senior technical product manager at GE Healthcare. The other authors have no conflicts of interest to declare.
Ethical Statement: The authors are accountable for all aspects of the work in ensuring that questions related to the accuracy or integrity of any part of the work are appropriately investigated and resolved. This study was exempt by the institutional review board and the informed consent due to its retrospective nature. And all procedures performed in this study were in accordance with the Declaration of Helsinki (as revised in 2013).
Open Access Statement: This is an Open Access article distributed in accordance with the Creative Commons Attribution-NonCommercial-NoDerivs 4.0 International License (CC BY-NC-ND 4.0), which permits the non-commercial replication and distribution of the article with the strict proviso that no changes or edits are made and the original work is properly cited (including links to both the formal publication through the relevant DOI and the license). See: https://creativecommons.org/licenses/by-nc-nd/4.0/.
References
- Ricardi U, Badellino S, Filippi AR. Stereotactic body radiotherapy for early stage lung cancer: history and updated role. Lung Cancer 2015;90:388-96. [Crossref] [PubMed]
- Ceniceros L, Aristu J, Castanon E, et al. Stereotactic body radiotherapy (SBRT) for the treatment of inoperable stage I non-small cell lung cancer patients. Clin Transl Oncol 2016;18:259-68. [Crossref] [PubMed]
- Taremi M, Hope A, Dahele M, et al. Stereotactic body radiotherapy for medically inoperable lung cancer: prospective, single-center study of 108 consecutive patients. Int J Radiat Oncol Biol Phys 2012;82:967-73. [Crossref] [PubMed]
- Videtic GM, Donington J, Giuliani M, et al. Stereotactic body radiation therapy for early-stage non-small cell lung cancer: Executive Summary of an ASTRO Evidence-Based Guideline. Pract Radiat Oncol 2017;7:295-301. [Crossref] [PubMed]
- Woody NM, Stephans KL, Andrews M, et al. A histologic basis for the efficacy of SBRT to the lung. J Thorac Oncol 2017;12:510-9. [Crossref] [PubMed]
- British Thoracic Society Society of Cardiothoracic Surgeons of Great Britain Ireland Working Party. Guidelines on the selection of patients with lung cancer for surgery. Thorax 2001;56:89-108. [Crossref] [PubMed]
- Puri V, Crabtree TD, Bell JM, et al. National cooperative group trials of "high-risk" patients with lung cancer: are they truly "high-risk"? Ann Thorac Surg 2014;97:1678-83; discussion 1683-5. [Crossref] [PubMed]
- Sancheti MS, Melvan JN, Medbery RL, et al. Outcomes after surgery in high-risk patients with early stage lung cancer. Ann Thorac Surg. 2016;101:1043-50; discussion 1051. [Crossref] [PubMed]
- Heerink WJ, de Bock GH, de Jonge GJ, et al. Complication rates of CT-guided transthoracic lung biopsy: meta-analysis. Eur Radiol 2017;27:138-48. [Crossref] [PubMed]
- Wiener RS, Wiener DC, Gould MK. Risks of transthoracic needle biopsy: how high? Clin Pulm Med 2013;20:29-35. [Crossref] [PubMed]
- Boskovic T, Stanic J, Pena-Karan S, et al. Pneumothorax after transthoracic needle biopsy of lung lesions under CT guidance. J Thorac Dis 2014;6:S99-107. [PubMed]
- Wiener RS, Schwartz LM, Woloshin S, et al. Population-based risk for complications after transthoracic needle lung biopsy of a pulmonary nodule: an analysis of discharge records. Ann Intern Med 2011;155:137-44. [Crossref] [PubMed]
- Yildirim E, Kirbas I, Harman A, et al. CT-guided cutting needle lung biopsy using modified coaxial technique: factors effecting risk of complications. Eur J Radiol 2009;70:57-60. [Crossref] [PubMed]
- Manhire A, Charig M, Clelland C, et al. Guidelines for radiologically guided lung biopsy. Thorax 2003;58:920-36. [Crossref] [PubMed]
- Wahidi MM, Rocha AT, Hollingsworth JW, et al. Contraindications and safety of transbronchial lung biopsy via flexible bronchoscopy. A survey of pulmonologists and review of the literature. Respiration 2005;72:285-95. [Crossref] [PubMed]
- Adeloye D, Chua S, Lee C, et al. Global and regional estimates of COPD prevalence: systematic review and meta-analysis. J Glob Health 2015;5:020415. [Crossref] [PubMed]
- Walser T, Cui X, Yanagawa J, et al. Smoking and lung cancer: the role of inflammation. Proc Am Thorac Soc 2008;5:811-5. [Crossref] [PubMed]
- Wang W, Dou S, Dong W, et al. Impact of COPD on prognosis of lung cancer: from a perspective on disease heterogeneity. Int J Chron Obstruct Pulmon Dis 2018;13:3767-76. [Crossref] [PubMed]
- Dai J, Yang P, Cox A, et al. Lung cancer and chronic obstructive pulmonary disease: From a clinical perspective. Oncotarget 2017;8:18513-24. [Crossref] [PubMed]
- Charlson ME, Pompei P, Ales KL, et al. A new method of classifying prognostic comorbidity in longitudinal studies: development and validation. J Chronic Dis 1987;40:373-83. [Crossref] [PubMed]
- Quan H, Li B, Couris CM, et al. Updating and validating the Charlson comorbidity index and score for risk adjustment in hospital discharge abstracts using data from 6 countries. Am J Epidemiol 2011;173:676-82. [Crossref] [PubMed]
- Radovanovic D, Seifert B, Urban P, et al. Validity of Charlson Comorbidity Index in patients hospitalised with acute coronary syndrome. Insights from the nationwide AMIS Plus registry 2002-2012. Heart 2014;100:288-94. [Crossref] [PubMed]
- Bøje CR, Dalton SO, Primdahl H, et al. Evaluation of comorbidity in 9388 head and neck cancer patients: a national cohort study from the DAHANCA database. Radiother Oncol 2014;110:91-7. [Crossref] [PubMed]
- Yang CC, Fong Y, Lin LC, et al. The age-adjusted Charlson comorbidity index is a better predictor of survival in operated lung cancer patients than the Charlson and Elixhauser comorbidity indices. Eur J Cardiothorac Surg 2018;53:235-40. [Crossref] [PubMed]
- Robbins JR, Gayar OH, Zaki M, et al. Impact of age-adjusted Charlson comorbidity score on outcomes for patients with early-stage endometrial cancer. Gynecol Oncol 2013;131:593-7. [Crossref] [PubMed]
- Suzuki H, Hanai N, Nishikawa D, et al. The Charlson comorbidity index is a prognostic factor in sinonasal tract squamous cell carcinoma. Jpn J Clin Oncol 2016;46:646-51. [Crossref] [PubMed]
- Yang CC, Chen PC, Hsu CW, et al. Validity of the age-adjusted charlson comorbidity index on clinical outcomes for patients with nasopharyngeal cancer post radiation treatment: a 5-year nationwide cohort study. PLoS One 2015;10:e0117323. [Crossref] [PubMed]
- Manig L, Käsmann L, Janssen S, et al. Simplified comorbidity score and Eastern Cooperative Oncology Group performance score predicts survival in patients receiving organ-preserving treatment for bladder cancer. Anticancer Res 2017;37:2693-6. [Crossref] [PubMed]
- Hochhegger B, Alves GR, Irion KL, et al. PET/CT imaging in lung cancer: indications and findings. J Bras Pneumol 2015;41:264-74. [Crossref] [PubMed]
- Gallamini A, Zwarthoed C, Borra A. Positron emission tomography (PET) in oncology. Cancers 2014;6:1821-89. [Crossref] [PubMed]
- Deppen SA, Blume JD, Kensinger CD, et al. Accuracy of FDG-PET to diagnose lung cancer in areas with infectious lung disease: a meta-analysis. JAMA 2014;312:1227-36. [Crossref] [PubMed]
- Croft DR, Trapp J, Kernstine K, et al. FDG-PET imaging and the diagnosis of non-small cell lung cancer in a region of high histoplasmosis prevalence. Lung Cancer 2002;36:297-301. [Crossref] [PubMed]
- Osmonov DK, Heimann D, Janßen I, et al. Sensitivity and specificity of PET/CT regarding the detection of lymph node metastases in prostate cancer recurrence. Springerplus 2014;3:340. [Crossref] [PubMed]
- Hong S, Li J, Wang S. 18FDG PET-CT for diagnosis of distant metastases in breast cancer patients. A meta-analysis. Surg Oncol 2013;22:139-43. [Crossref] [PubMed]
- Kim SK, Allen-Auerbach M, Goldin J, et al. Accuracy of PET/CT in characterization of solitary pulmonary lesions. J Nucl Med 2007;48:214-20. [PubMed]
- Harkenrider MM, Bertke MH, Dunlap NE. Stereotactic body radiation therapy for unbiopsied early-stage lung cancer: a multi-institutional analysis. Am J Clin Oncol 2014;37:337-42. [Crossref] [PubMed]
- Hasan S, Colonias A, Mickus T, et al. Image‐based management of empiric lung stereotactic body radiotherapy (SBRT) without biopsy: predictors from a 10‐year single institution experience. Thorac Cancer 2018;9:699-706. [Crossref] [PubMed]
- Wang Z, Li AM, Gao J, et al. Clinical outcomes of CyberKnife stereotactic radiosurgery for elderly patients with presumed primary stage I lung cancer. Transl Lung Cancer Res 2017;6:6-13. [Crossref] [PubMed]
- Inoue T, Shimizu S, Onimaru R, et al. Clinical outcomes of stereotactic body radiotherapy for small lung lesions clinically diagnosed as primary lung cancer on radiologic examination. Int J Radiat Oncol Biol Phys 2009;75:683-7. [Crossref] [PubMed]
- Sakanaka K, Matsuo Y, Nagata Y, et al. Safety and effectiveness of stereotactic body radiotherapy for a clinically diagnosed primary stage I lung cancer without pathological confirmation. Int J Clin Oncol 2014;19:814-21. [Crossref] [PubMed]
- Yoshitake T, Nakamura K, Shioyama Y, et al. Stereotactic body radiation therapy for primary lung cancers clinically diagnosed without pathological confirmation: a single-institution experience. Int J Clin Oncol 2015;20:53-8. [Crossref] [PubMed]
- Guckenberger M, Allgäuer M, Appold S, et al. Safety and efficacy of stereotactic body radiotherapy for stage I non-small-cell lung cancer in routine clinical practice: a patterns-of-care and outcome analysis. J Thorac Oncol 2013;8:1050-8. [Crossref] [PubMed]
- Valdes G, Solberg TD, Heskel M, et al. Using machine learning to predict radiation pneumonitis in patients with stage I non-small cell lung cancer treated with stereotactic body radiation therapy. Phys Med Biol 2016;61:6105-20. [Crossref] [PubMed]
- Kowalchuk RO, Trifiletti DM, Khandelwal SR, et al. A risk model for lung complication combining radiation therapy and chronic obstructive pulmonary disease. J Radiat Oncol 2019;8:209-16. [Crossref]
- Timmerman R, Paulus R, Galvin J, et al. Stereotactic body radiation therapy for inoperable early stage lung cancer. JAMA 2010;303:1070-6. [Crossref] [PubMed]
- Onishi H, Shirato H, Nagata Y, et al. Hypofractionated stereotactic radiotherapy (HypoFXSRT) for stage I non-small cell lung cancer: updated results of 257 patients in a Japanese multi-institutional study. J Thorac Oncol 2007;2:S94-100. [Crossref] [PubMed]
- Takeda A, Kunieda E, Sanuki N, et al. Stereotactic body radiotherapy (SBRT) for solitary pulmonary nodules clinically diagnosed as lung cancer with no pathological confirmation: comparison with non-small-cell lung cancer. Lung Cancer 2012;77:77-82. [Crossref] [PubMed]
- Haidar YM, Rahn DA 3rd, Nath S, et al. Comparison of outcomes following stereotactic body radiotherapy for non-small cell lung cancer in patients with and without pathological confirmation. Ther Adv Respir Dis 2014;8:3-12. [Crossref] [PubMed]
- Verstegen NE, Lagerwaard FJ, Haasbeek CJ, et al. Outcomes of stereotactic ablative radiotherapy following a clinical diagnosis of stage I NSCLC: comparison with a contemporaneous cohort with pathologically proven disease. Radiother Oncol 2011;101:250-4. [Crossref] [PubMed]
- Berman AT, Jabbour SK, Vachani A, et al. Empiric Radiotherapy for Lung Cancer Collaborative Group multi-institutional evidence-based guidelines for the use of empiric stereotactic body radiation therapy for non-small cell lung cancer without pathologic confirmation. Transl Lung Cancer Res 2019;8:5-14. [Crossref] [PubMed]